Chapter 8: Energy from Nutrients
8.2 Metabolism Basics
In this section we’ll introduce some basic concepts that will help you understand the following discussion of cellular metabolism. If you do not have much chemistry background, you may find it helpful to review Appendix C: Chemistry for Nutrition.
Metabolic Pathways
Consider the metabolism (both the creation and breakdown) of sugar. This is a classic example of one of the many cellular processes that use and produce energy. Living things consume sugars as a major energy source, because sugar molecules have a great deal of energy stored within their bonds. For the most part, photosynthesizing organisms like plants produce these sugars. During photosynthesis, plants use energy (originally from sunlight) to convert carbon dioxide gas (CO2) into sugar molecules (like glucose: C6H12O6). They consume carbon dioxide and produce oxygen as a waste product. This reaction is summarized as:
6CO2+ 6H2O–>C6H12O6+ 6O2
Because this process involves synthesizing an energy-storing molecule, it requires energy input to proceed. During photosynthesis, the energy from the sun is stored within molecules of adenosine triphosphate (ATP), which is the primary energy currency of all cells. Just as the dollar is used as currency to buy goods, cells use molecules of ATP as energy currency to perform immediate work. Energy-storage molecules such as glucose and fat are consumed so that they can be broken down to use their energy. The reaction that harvests the energy of a sugar molecule in cells requiring oxygen to survive can be summarized by the reverse reaction to photosynthesis. In this reaction, oxygen is consumed and carbon dioxide is released as a waste product. The reaction is summarized as:
C6H12O6+ 6O2–>6H2O + 6CO2
Both of these reactions involve many steps.
The processes of making and breaking down sugar molecules illustrate two examples of metabolic pathways. A metabolic pathway is a series of chemical reactions that takes a starting molecule and modifies it, step-by-step, through a series of metabolic intermediates, eventually yielding a final product. In the example of sugar metabolism, the first metabolic pathway synthesized sugar from smaller molecules, and the other pathway broke sugar down into smaller molecules. These two opposite processes—the first requiring energy and the second producing energy—are referred to as anabolic pathways (building polymers) and catabolic pathways (breaking down polymers into their monomers), respectively. Consequently, metabolism is composed of synthesis (anabolism) and degradation (catabolism) (Figure 8.21).
It is important to know that the chemical reactions of metabolic pathways do not take place on their own. Each reaction step is facilitated, or catalyzed, by a protein called an enzyme. Enzymes are important for catalyzing all types of biological reactions—those that require energy as well as those that release energy.
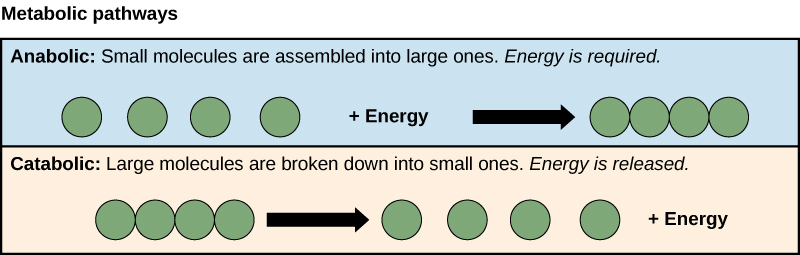
Energy metabolism refers more specifically to the metabolic pathways that release or store energy. Some of these are catabolic pathways, like glycolysis (the splitting of glucose), β-oxidation (fatty-acid breakdown), and amino acid catabolism. Others are anabolic pathways, and include those involved in storing excess energy (such as glycogenesis), and synthesizing triglycerides (lipogenesis). The table below summarizes some of the catabolic and anabolic pathways and their functions in energy metabolism.
Table 8.21 Metabolic Pathways[1]
Catabolic Pathway | Function | Anabolic Pathway | Function |
Glycolysis | Glucose breakdown | Gluconeogenesis | Synthesize glucose |
Glycogenolysis | Glycogen breakdown | Glycogenesis | Synthesize glycogen |
β-oxidation | Fatty-acid breakdown | Lipogenesis | Synthesize triglycerides |
Proteolysis | Protein breakdown to amino acids | Protein synthesis | Synthesize proteins |
Oxidation-Reduction Reactions[2]
A number of the metabolic reactions either oxidize or reduce compounds. A compound that is being oxidized loses at least one electron, while a compound that is reduced gains at least one electron. To remember the difference, a mnemonic device such as OIL (oxidation is lost), RIG (reduction is gained) is helpful. Oxidation reactions and reduction reactions are “coupled” reactions, one cannot exist without the other. For example, a reduction reaction requires an electron. Where does that electron come from? It comes from an oxidation reaction. Scientists commonly refer to oxidation reactions and reduction reactions as oxidation-reduction reactions, or as redox reactions. Oxidation-reduction reactions are illustrated in the figure below.
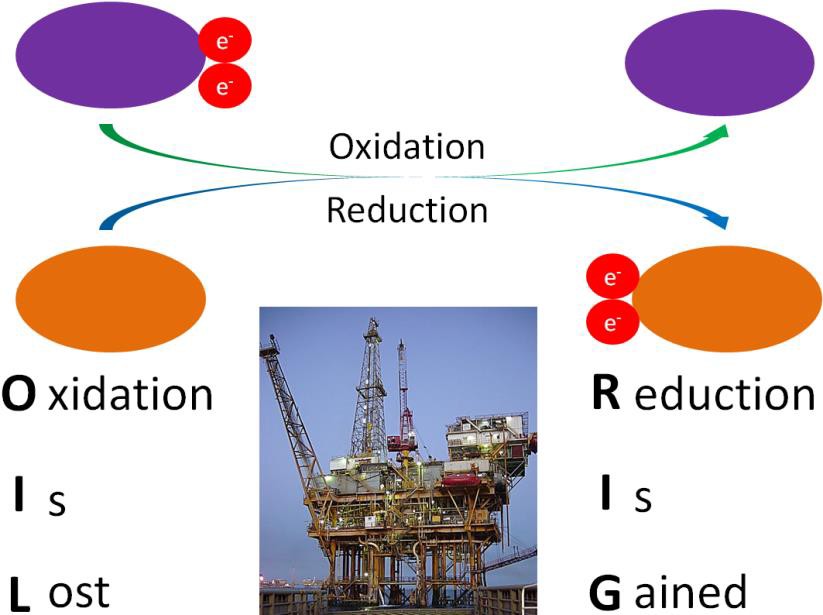
Another way to remember oxidation versus reduction is LEO goes GER (like a lion)
Lose Elections = Oxidation
Gain Elections = Reduction (YES, gaining electrons is considered reduction)
Iron is a good example we can use to illustrate oxidation-reduction reactions. Iron commonly exists in two states (Fe3+ or Fe2+). It is constantly oxidized/reduced back and forth between the two states. The oxidation/reduction of iron is shown below.
Fe3+ loses an e- → Fe2+ (Oxidation) Fe2+ gains an e- → Fe3+ (Reduction)
Interestingly, the oxidation states of iron (mentioned above) are critical to our ability to use the iron present in our diet. Fe2+ (also known as ferrous iron) is easily absorbed in the small intestine. Fe3+ (also known as ferric iron) is not so easily absorbed. Gastric acid (produced by the stomach) and vitamin C promote the conversion of Fe3+ to Fe2+ so we can maximize iron absorption in the small intestine.
However, some oxidation reduction reactions are not as easy to recognize. There are some simple rules to help you recognize less-obvious oxidation/reduction reactions that are based upon the gain or loss of oxygen or hydrogen. These are as follows:
Oxidation: gains oxygen or loses hydrogen
Reduction: loses oxygen or gains hydrogen
Remembering how this applies to hydrogen will be very helpful later in this chapter.
Enzymes[3]
We briefly discussed enzymes earlier when learning about the functions of proteins in the body. We will review enzymes here and go into a bit more detail.
A substance that helps a chemical reaction to occur is called a catalyst, and the molecules that catalyze biochemical reactions are called enzymes. Most enzymes are proteins and perform the critical task of lowering the activation energies of chemical reactions inside the cell. Most of the reactions critical to a living cell happen too slowly at normal temperatures to be of any use to the cell. Without enzymes to speed up these reactions, life could not persist. Enzymes do this by binding to the reactant molecules and holding them in such a way as to make the chemical bond-breaking and -forming processes take place more easily. An enzyme itself is unchanged by the reaction it catalyzes. Once one reaction has been catalyzed, the enzyme is able to participate in other reactions.
The chemical reactants to which an enzyme binds are called the enzyme’s substrates. There may be one or more substrates, depending on the particular chemical reaction. In some reactions, a single reactant substrate is broken down into multiple products. In others, two substrates may come together to create one larger molecule. Two reactants might also enter a reaction and both become modified, but they leave the reaction as two products. The location within the enzyme where the substrate binds is called the enzyme’s active site. The active site is where the “action” happens. Since enzymes are proteins, there is a unique combination of amino acid side chains within the active site. Each side chain is characterized by different properties. They can be large or small, weakly acidic or basic, hydrophilic or hydrophobic, positively or negatively charged, or neutral. The unique combination of side chains creates a very specific chemical environment within the active site. This specific environment is suited to bind to one specific chemical substrate (or substrates).
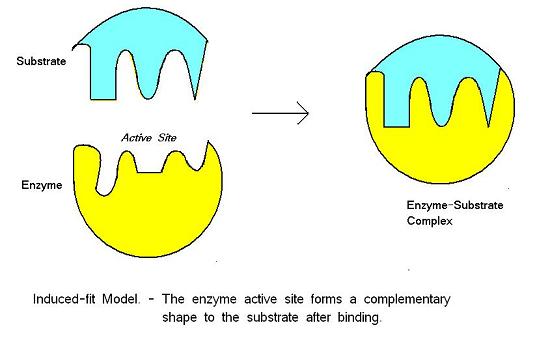
For many years, scientists thought that enzyme-substrate binding took place in a simple “lock and key” fashion. This model asserted that the enzyme and substrate fit together perfectly in one instantaneous step. However, current research supports a model called induced fit (Figure 8.24). The induced-fit model expands on the lock-and-key model by describing a more dynamic binding between enzyme and substrate. As the enzyme and substrate come together, their interaction causes a slight shift in the enzyme’s structure that forms an ideal binding arrangement between enzyme and substrate.
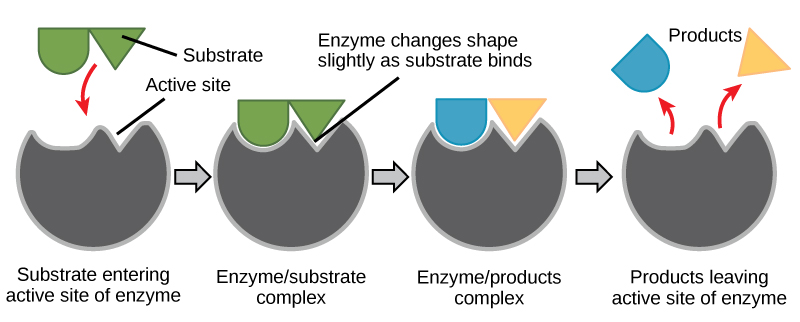
When an enzyme binds its substrate, an enzyme-substrate complex is formed. This complex lowers the activation energy of the reaction and promotes its rapid progression in one of multiple possible ways.
- On a basic level, enzymes promote chemical reactions that involve more than one substrate by bringing the substrates together in an optimal orientation for reaction.
- Enzymes promote the reaction of their substrates is by creating an optimal environment within the active site for the reaction to occur. The chemical properties that emerge from the particular arrangement of amino acid R groups (side chains) within an active site create the perfect environment for an enzyme’s specific substrates to react.
- The enzyme-substrate complex can also lower activation energy by compromising the bond structure so that it is easier to break.
- Finally, enzymes can also lower activation energies by taking part in the chemical reaction itself. In these cases, it is important to remember that the enzyme will always return to its original state by the completion of the reaction.
One of the hallmark properties of enzymes is that they remain ultimately unchanged by the reactions they catalyze. After an enzyme has catalyzed a reaction, it releases its product(s) and can catalyze a new reaction.
Cofactors[4]
A number of enzymes require cofactors to function. Cofactors can be either organic or inorganic molecules that are required by enzymes to function. Many organic cofactors are vitamins or molecules derived from vitamins. Most inorganic cofactors are minerals. Cofactors can be oxidized or reduced for the enzymes to catalyze the reactions. Organic cofactors are often referred to as coenzymes.
Two common cofactors that are derived from the B vitamins, niacin and riboflavin, are NAD (nicotinamide adenine dinucleotide) and FAD (flavin adenine dinucleotide), respectively.
Both of these cofactors can be reduced (remember that reduction is a process by which electrons, as part of H in this case, are gained); NAD is reduced to form NADH, while FAD is reduced to form FADH2 as shown in the two figures below.
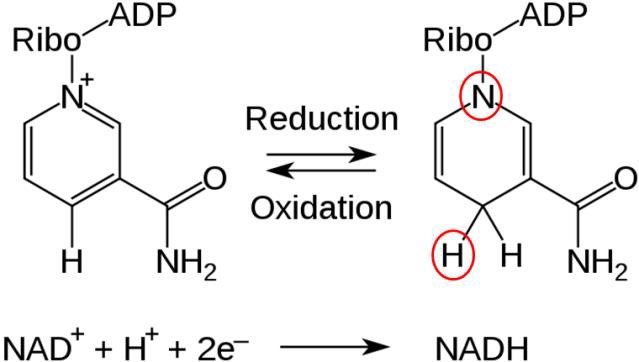
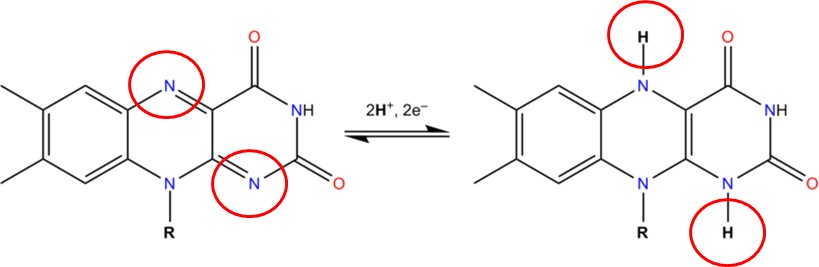
NADH and FADH2 are molecules that are critical to our cells’ ability to process the energized electrons obtained through the catabolism (digestion) of food molecules, like glucose. The energized electrons, which are highly reactive and potentially destructive, are temporarily managed by NADH and FADH2 until they can be processed by the Electron Transport Chain step of Cellular Respiration.
Together, all of the chemical reactions that take place inside cells, including those that consume or generate energy, are referred to as the cell’s metabolism.
Photosynthesis is the process by which plants use energy from sunlight to convert carbon dioxide gas (CO2) from the atmosphere into sugar molecules, like glucose.
Anabolism refers to anabolic metabolic pathways. Anabolic pathways use energy to build polymers from smaller molecules.
Catabolism refers to catabolic metabolic pathways. Catabolic pathways break down polymers into their monomers, releasing energy
Amino acids are the building blocks of proteins, simple subunits composed of carbon, oxygen, hydrogen, and nitrogen.
Triglycerides are the main form of lipid found in the body and in the diet. A triglyceride molecule consists of a glycerol backbone attached to three fatty acids.
A compound is reduced when it gains at least one electron.
"Redox reactions" refers to paired oxidation and reduction reactions together.
The location within the enzyme where the substrate binds is called the enzyme’s active site.
Vitamins are organic compounds that must be taken in from the diet.
Minerals are solid inorganic substances that form crystals and are classified depending on how much of them we need. Trace minerals such as zinc, iron, or iodine are only required in a few milligrams or less per day. Major minerals such as calcium, sodium, and potassium are required in hundreds of milligrams per day.